Status and pace of progress
Heating and cooling accounts for about half of the global final energy consumption. It is the largest source of energy end use, ahead of electricity (20%) and transport (30%), and is responsible for more than 40% of global energy-related carbon dioxide emissions.
The majority of heat generation comes from fossil fuels (coal, oil and natural gas), with some from the unsustainable use of biomass. The share of fossil fuels in heat generation has, nevertheless, been declining (from 91% in 2010 to 75% in 2021) (IEA, 2022a). Meanwhile, the buildings (residential and commercial) and industrial sectors account for approximately 95% of global heating demand.
The global energy demand for cooling is expected to increase by 45% by 2050 compared with 2016 levels (from 7 to 12 exajoules [EJ]) (IEA, 2018). One reason for this is that only a third of the global population currently living in hot climates possesses cooling appliances (Camarasa et al., 2022). Another reason is that global temperatures are rising, with an increase of 1.5°C or more over pre-industrial levels expected by 2035 (IPCC, 2018). Both reasons are likely to cause an increase in the demand for cooling appliances, especially with rising standards of living in developing countries. For example, the number of cooling units in China and India increased by more than 60% between 2010 and 2020, and is expected to increase threefold in the current decade (Green Cooling Initiative, 2022).
Space cooling, mostly in buildings, is responsible for roughly half of the energy used for cooling. The rest of the energy is used for industrial and commercial refrigeration (Green Cooling Initiative, 2022). Since cooling is already predominantly powered by electricity, decarbonisation gains will be linked to smart electrification strategies rather than greater electrification, as in the case of heat.
Electrification, primarily of heating, is the key strategy for decarbonising heating and cooling (and meeting the 1.5°C target by 2050). 1 IRENA’s 1.5°C Scenario shows that by 2050, electricity would power 73% of the total demand in buildings, up from the current 34%. This will require putting 793 million heat pump units into operation by 2050, a 14-fold increase from today’s 58 million units. In the industrial sector, some industrial processes, such as steel industry furnaces, are difficult to electrify. Nonetheless, by 2050, electricity should account for 27% of total final energy consumption in industry (IRENA, 2023). Figure 4.1 shows the projections for 2030 and 2050 under IRENA’s 1.5°C Scenario.
The heating (and cooling 2 ) sector is divided into two end uses, buildings and industry, since industry often requires much higher temperatures (>150°C) than buildings (<100°C). Residential and commercial buildings can also be divided into those with small-scale heating and cooling systems or large-scale district heating systems. Each end use has a different current status and pace of progress and will thus require different innovations.
For example, district heating and cooling (DHC) systems still predominantly rely on fossil fuels (90% of the total heating supply), even though they could switch to renewable sources, waste heat streams or other sources (IEA, 2019). The use of district heating has increased 32% since 2010, accounting for 16 EJ of heating world-wide in 2021. District cooling (now only 1-2% of the entire European cooling market) is expected to increase by 3.5% annually over the next five years (Research and Markets, 2022).
For all buildings globally, 62% of heating was supplied by fossil fuels in 2020, with the remainder coming from traditional uses of biomass (26%) and modern renewables (12%). 3 The renewables share is expected to increase rapidly.
The industrial sector uses both low-temperature heat (for example, food and chemicals require less than 100°C) and high-temperature heat (steel, cement and glass industries require temperatures above 1 000°C), and predominantly relies on fossil fuels (89% in 2020). High-temperature processes (those that require temperatures above the range of heat pumps’, greater than 150°C) are difficult to electrify directly at high efficiencies. These processes can alternatively be indirectly electrified using “green” hydrogen (see Section III).
FIGURE 4.1 Electricity shares and market roll-out of heat pumps under IRENA’s 1.5°C Scenario for the industry and buildings sectors
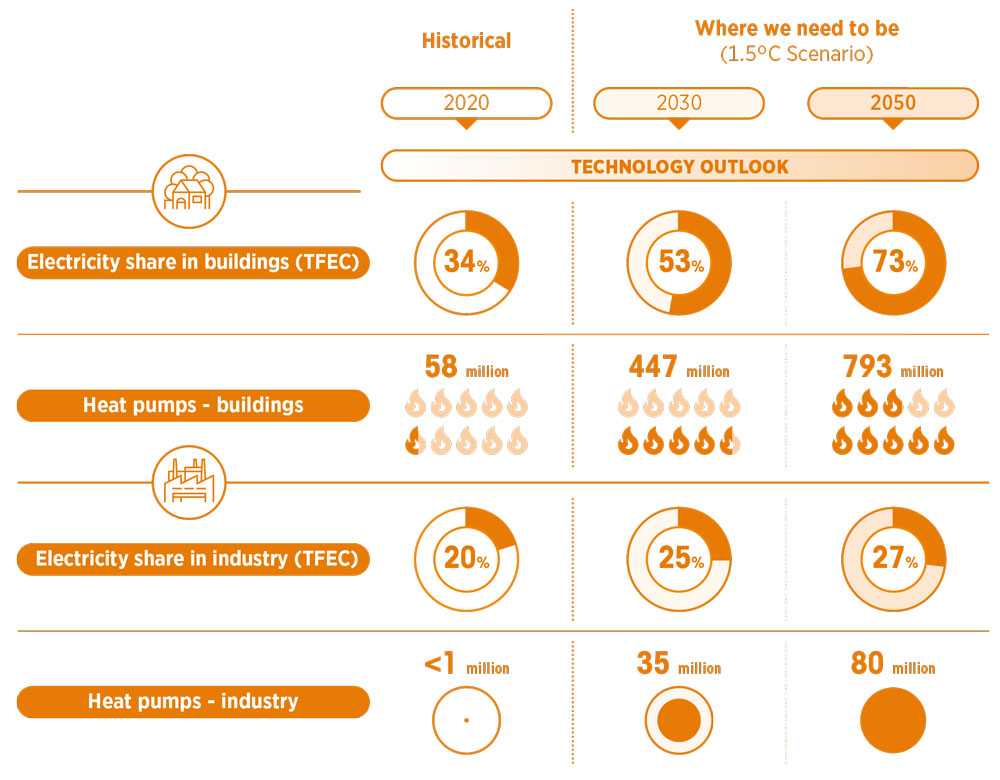
Source: TFEC = total final energy consumption.
BOX 4.1 Success factors for the deployment of large-scale heat pumps: The Swedish case
Sweden’s electricity generation far exceeded demand, thanks to its extensive hydropower resources and the construction of nuclear power plants in the 1970s and 1980s. The surpluses could not be exported, however, due to the limited transmission capacity with neighbouring countries. To use some of the surplus electricity, Sweden developed district heating systems with large heat pumps, installing a total capacity of 1 527 MW in the 1980s. Eighty percent of that capacity is still in use.
FIGURE 4.2 Annual proportion of heat supplied to Swedish district heating systems from electric boilers and heat pumps, 1970-2013
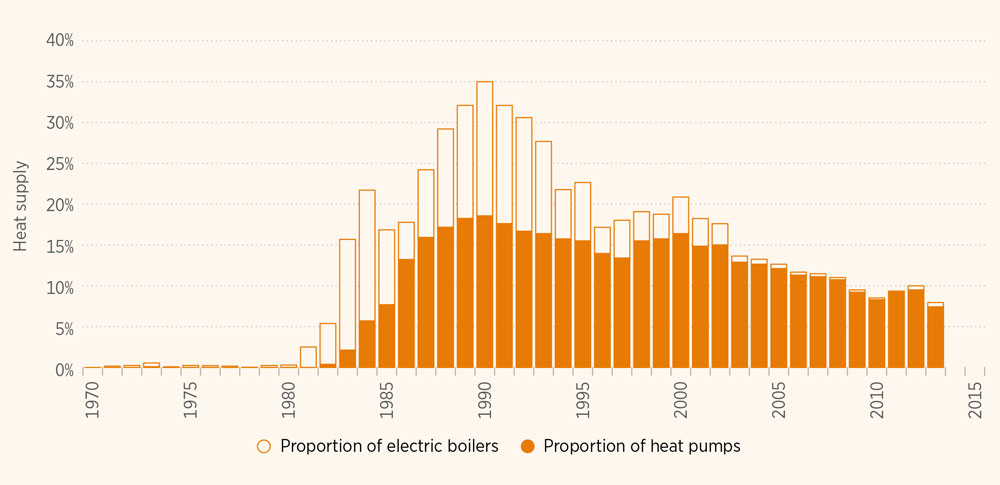
After 2000, however, combined heat and power plants became more cost-competitive with heat pumps due to higher electricity prices and taxes. This led to a decline in the share of heat from heat pumps (Figure 4.2). Nevertheless, the Swedish example includes valuable operational and maintenance experience, including the adoption of new refrigerants and the expansion of the use of heat pumps to a larger range of temperatures and applications, especially in the industrial sector.
4.1 Importance of smart electrification for future heating and cooling systems
The smart electrification strategies discussed in this report seek to create heating and cooling systems that are more efficient, effective, reliable and secure. They make it possible to integrate more renewable sources, reduce peak loads and congestion, and, ultimately, sharply reduce the dependence on fossil fuels, while also reducing costs and environmental impacts. They can also add flexibility to the power system, making it more efficient, avoiding investments in additional generation capacity in transmission or distribution grids.
Smart electrification strategies are heavily context dependent, since there is no simple guaranteed solution that works for all. The initiatives listed below are examples of successful cases that support smart electrification strategies in different countries:
- Smart electrification via remote-controlled heating and cooling systems: Predictive and adaptive algorithms make it possible to adjust heating, ventilation, cooling or refrigeration based on real-time power market conditions and consumers’ needs, thus ensuring comfort at lower prices. As an example, in France, BeeBryte is already selling such remote-control services (BeeBright, n.d.).
- Thermal storage makes it possible to integrate more renewable sources. Storing just one cubic metre (m3) of water-based heat can facilitate a 55-70% increase in the share of cooling demand that can be met by solar photovoltaic (PV) (Laine et al., 2019). In Nigeria, for example, proposed ice-based energy storage with solar PV-powered refrigeration can reduce energy consumption by up to 40% in cooling-as-a-service models (Koolboks, 2020).
- Aggregating decentralised distributed energy resources enables them to provide flexibility services to grids. For example, in Belgium, demand-response operations using 40 000 residential heat pumps can provide 100 MW of upward reserve 4 at a cost of EUR 0-14/MWh, much lower than the local historical price of EUR 32/MWh (Georges et al., 2017). The refrigeration units in supermarkets also offer several megawatts of flexible load. Because they can adjust cooling load by 60-80% very quickly, they can provide grid-balancing services (Danfoss, 2017). In another example, Japanese manufacturer Daikin and Next Kraftwerke, a German aggregator, have demonstrated that air-conditioning systems with cold storage tanks can be aggregated into virtual power plants (NEDO, 2018).
- Smart electrification of industrial heating demand: Pilot projects have demonstrated that the heat demand of aluminium smelting plants could be modulated up or down by 25% for up to 48 hours. This flexibility, coupled with on-site wind generation, has increased the share of renewable energy used in smelter plants by up to 50% (TRIMET, 2021).
- Waste heat recovery: Reuse of industrial waste heat has enabled an Irish dairy product factory to reduce its energy consumption by 12%, helping it to save EUR 150 000 annually. This has also enabled a chocolate factory in the Netherlands to cut energy use by 6%. Another source of useful heat is city sewage systems. Cologne, Germany, recovered 600 MWh of thermal energy from its sewage system in 2017 (5% of the total city demand) (Celsius, 2020).
- Natural cooling sources: According to Enware, the company owning and operating Toronto’s deep lake water cooling (DLWC) system, this Canadian city saves 90 TWh of electricity annually by using cold water from the depths of Lake Ontario to cool more than 100 buildings. These savings are equivalent to the electricity consumption of a town of 25 000 inhabitants (City of Toronto, 2021).